تاريخ الفيزياء
علماء الفيزياء
الفيزياء الكلاسيكية
الميكانيك
الديناميكا الحرارية
الكهربائية والمغناطيسية
الكهربائية
المغناطيسية
الكهرومغناطيسية
علم البصريات
تاريخ علم البصريات
الضوء
مواضيع عامة في علم البصريات
الصوت
الفيزياء الحديثة
النظرية النسبية
النظرية النسبية الخاصة
النظرية النسبية العامة
مواضيع عامة في النظرية النسبية
ميكانيكا الكم
الفيزياء الذرية
الفيزياء الجزيئية
الفيزياء النووية
مواضيع عامة في الفيزياء النووية
النشاط الاشعاعي
فيزياء الحالة الصلبة
الموصلات
أشباه الموصلات
العوازل
مواضيع عامة في الفيزياء الصلبة
فيزياء الجوامد
الليزر
أنواع الليزر
بعض تطبيقات الليزر
مواضيع عامة في الليزر
علم الفلك
تاريخ وعلماء علم الفلك
الثقوب السوداء
المجموعة الشمسية
الشمس
كوكب عطارد
كوكب الزهرة
كوكب الأرض
كوكب المريخ
كوكب المشتري
كوكب زحل
كوكب أورانوس
كوكب نبتون
كوكب بلوتو
القمر
كواكب ومواضيع اخرى
مواضيع عامة في علم الفلك
النجوم
البلازما
الألكترونيات
خواص المادة
الطاقة البديلة
الطاقة الشمسية
مواضيع عامة في الطاقة البديلة
المد والجزر
فيزياء الجسيمات
الفيزياء والعلوم الأخرى
الفيزياء الكيميائية
الفيزياء الرياضية
الفيزياء الحيوية
الفيزياء العامة
مواضيع عامة في الفيزياء
تجارب فيزيائية
مصطلحات وتعاريف فيزيائية
وحدات القياس الفيزيائية
طرائف الفيزياء
مواضيع اخرى
Nuclear Models
المؤلف:
Roger J Blin-Stoyle, FRS
المصدر:
Physics of Particles, Matter and the Universe
الجزء والصفحة:
p 133
24-5-2016
4429
Nuclear Models
The extreme complexity of the nuclear force coupled with the fact that nuclei can contain up to 250 or more nucleons means that it is very difficult to develop an exact theoretical treatment of nuclear structure. The approach during the last 50 years has therefore been to devise conceptual models of the nucleus which are simpler to deal with but which are reasonably close to its physical structure and which enable quite detailed understanding of nuclear behaviour to be achieved. Such models tend to focus on different aspects of nuclear structure and on different groups of nuclei. One of the simplest and oldest models, which accounts for the general aspects of nuclear masses and binding energies, is known as the liquid drop model. This model is based on the similarity between a drop of liquid and an atomic nucleus. In a liquid drop the component atoms or molecules are held together by a force which is vaguely similar to, although of very different range and strength (see the previous section), to the nuclear force. For example, in both systems there is a net inward force on their components at the surface which results in a ‘surface tension’ effect. This constrains drops and nuclei to be roughly spherical in shape. By treating the nucleus as a liquid drop and including this surface tension effect together with an additional force that does not occur in a liquid drop, namely the electric repulsion between the protons, it becomes possible to account in reasonable detail for the shape of the B/A. Such a model is essentially classical, however, and therefore cannot help with understanding the energy levels in a nucleus. This was first achieved by the introduction of the nuclear shell model which is analogous in many ways to an atomic like description of the nucleus. Focusing attention on a single neutron as it moves through the nucleus there is, on average, no net force on it due to other nucleons whilst in the middle of the nucleus since they surround it fairly uniformly. It simply experiences a roughly constant potential energy due to them as shown in figure 1.1. However, as it moves towards the nuclear surface, there will be an increasing attractive inward force.
Figure 1.1: The average potential energy experienced by a neutron in a nucleus.
Leading to a rise in the potential energy. This eventually reduces to zero as the neutron moves away from the nucleus and out of the range of the nuclear force. The neutron is thus confined in a potential well similar in general features. Protons are similarly confined but the wells have a slightly different shape at the edges due to the additional electric force they experience. In such a well a series of different quantum energy levels will be available for the nucleons to occupy, each level being characterized by several quantum numbers in a similar fashion to the energy levels available to an electron in an atom. Because of the Pauli exclusion principle, each level will only be able to accommodate a certain number of neutrons and protons and, when full, we speak of filled shells as in the analogous atomic situation. These ‘fillings’ occur when the number of protons ( Z ) and the number of neutrons ( N ) take the following values (referred to as magic numbers).
Nuclei with filled shells are particularly stable. Examples are helium (Z = N = 2), oxygen (Z = N = 8) and lead (Z = 82, N = 126). These nuclei are in this respect somewhat like atoms of the inert gases. The energy levels and other (e.g. electromagnetic) properties of nuclei containing a few nucleons in excess of, or less than, magic numbers can then be very readily accounted for in considerable detail in terms of the behaviour of these ‘loose’ nucleons or holes-a nucleon missing from a shell corresponds to a hole in the shell. These interact with each other through, as it were, the remnants of the nuclear force not included in the average potential well. However when there are many loose nucleons the situation becomes extremely complicated and another approach to nuclear structure was developed the collective model. Loose nucleons tend to alter the nuclear shape and with many of them the nucleus can become quite distorted. If there are not too
Figure 1.2: Nuclear collective motion: (a) vibrational; (b) rotational.
many it is found that the whole nucleus oscillates in shape, for example, backwards and forwards from spherical (soccer ball) to ellipsoidal (American football) shape (see figure 1.2(a)). These oscillations are, of course, quantized and lead to a series of equally spaced oscillator energy levels, many examples of which have been observed. Another form of collective oscillation which also occurs is when the neutrons and protons oscillate en masse in opposite directions to each other. When the number of loose nucleons is very high nuclei become permamently deformed; in some extreme cases the length and width for an American football like shape are in the ratio 2:l. For such nuclei it is natural for them to rotate as shown in figure 1.2(b), leading to a series of quantized ‘rotational’ energy levels. Angular momenta as large as 60 h have been observed. For much larger values the nucleus will fly apart because of the centrifugal force. These different nuclear models have formed the basis for understanding many nuclear properties. They must all, in the deepest sense, be related to each other and much very complicated theoretical work is in progress now to clarify the situation.
الاكثر قراءة في مواضيع عامة في الفيزياء النووية
اخر الاخبار
اخبار العتبة العباسية المقدسة
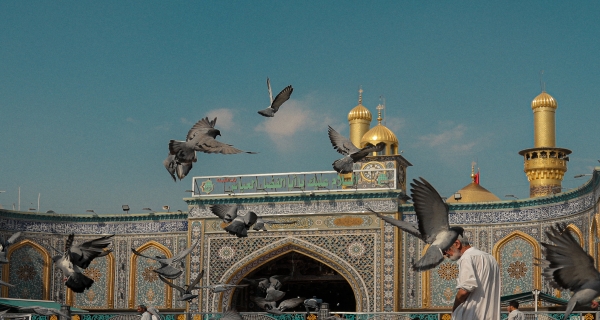
الآخبار الصحية
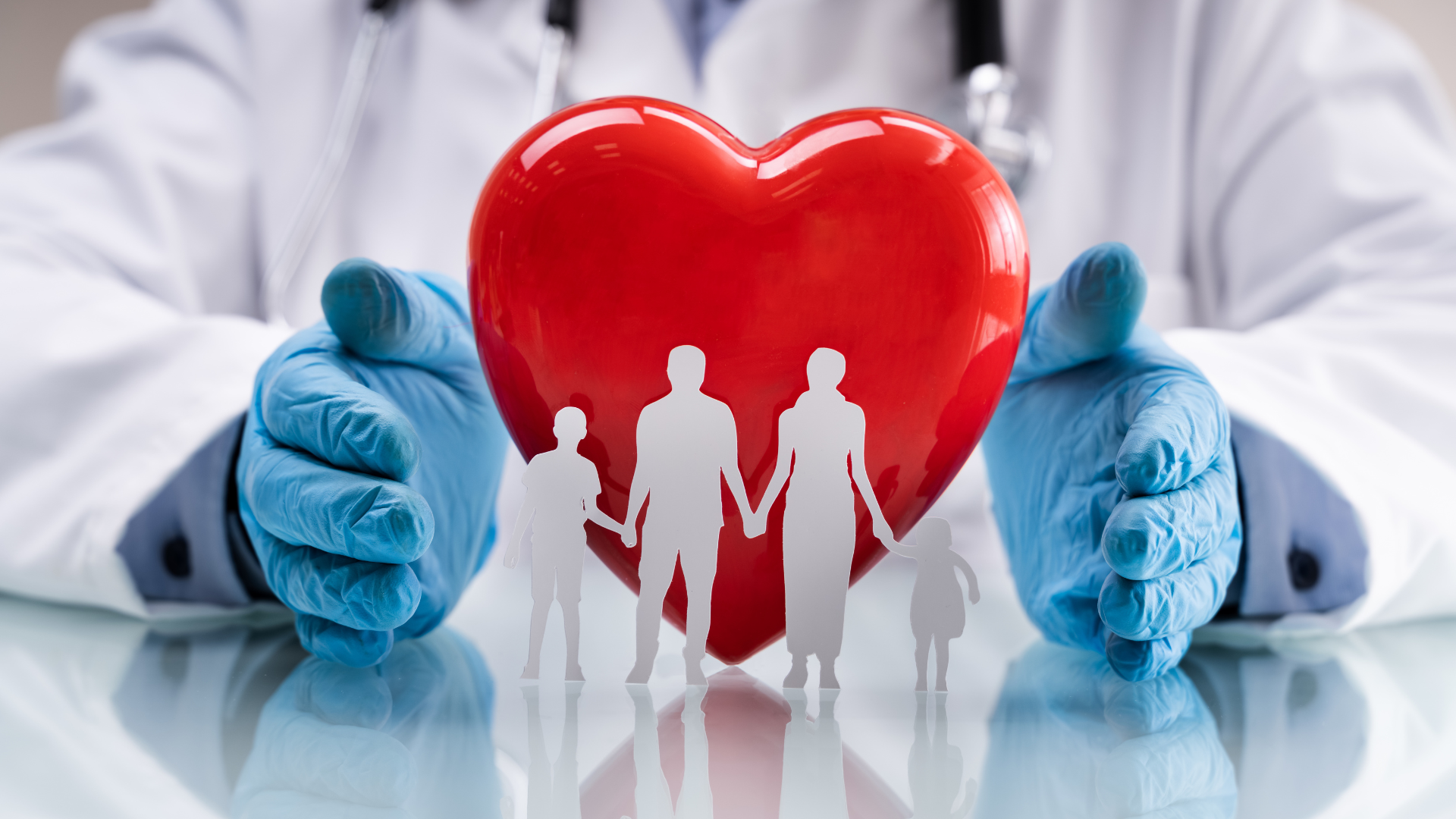