النبات
مواضيع عامة في علم النبات
الجذور - السيقان - الأوراق
النباتات الوعائية واللاوعائية
البذور (مغطاة البذور - عاريات البذور)
الطحالب
النباتات الطبية
الحيوان
مواضيع عامة في علم الحيوان
علم التشريح
التنوع الإحيائي
البايلوجيا الخلوية
الأحياء المجهرية
البكتيريا
الفطريات
الطفيليات
الفايروسات
علم الأمراض
الاورام
الامراض الوراثية
الامراض المناعية
الامراض المدارية
اضطرابات الدورة الدموية
مواضيع عامة في علم الامراض
الحشرات
التقانة الإحيائية
مواضيع عامة في التقانة الإحيائية
التقنية الحيوية المكروبية
التقنية الحيوية والميكروبات
الفعاليات الحيوية
وراثة الاحياء المجهرية
تصنيف الاحياء المجهرية
الاحياء المجهرية في الطبيعة
أيض الاجهاد
التقنية الحيوية والبيئة
التقنية الحيوية والطب
التقنية الحيوية والزراعة
التقنية الحيوية والصناعة
التقنية الحيوية والطاقة
البحار والطحالب الصغيرة
عزل البروتين
هندسة الجينات
التقنية الحياتية النانوية
مفاهيم التقنية الحيوية النانوية
التراكيب النانوية والمجاهر المستخدمة في رؤيتها
تصنيع وتخليق المواد النانوية
تطبيقات التقنية النانوية والحيوية النانوية
الرقائق والمتحسسات الحيوية
المصفوفات المجهرية وحاسوب الدنا
اللقاحات
البيئة والتلوث
علم الأجنة
اعضاء التكاثر وتشكل الاعراس
الاخصاب
التشطر
العصيبة وتشكل الجسيدات
تشكل اللواحق الجنينية
تكون المعيدة وظهور الطبقات الجنينية
مقدمة لعلم الاجنة
الأحياء الجزيئي
مواضيع عامة في الاحياء الجزيئي
علم وظائف الأعضاء
الغدد
مواضيع عامة في الغدد
الغدد الصم و هرموناتها
الجسم تحت السريري
الغدة النخامية
الغدة الكظرية
الغدة التناسلية
الغدة الدرقية والجار الدرقية
الغدة البنكرياسية
الغدة الصنوبرية
مواضيع عامة في علم وظائف الاعضاء
الخلية الحيوانية
الجهاز العصبي
أعضاء الحس
الجهاز العضلي
السوائل الجسمية
الجهاز الدوري والليمف
الجهاز التنفسي
الجهاز الهضمي
الجهاز البولي
المضادات الحيوية
مواضيع عامة في المضادات الحيوية
مضادات البكتيريا
مضادات الفطريات
مضادات الطفيليات
مضادات الفايروسات
علم الخلية
الوراثة
الأحياء العامة
المناعة
التحليلات المرضية
الكيمياء الحيوية
مواضيع متنوعة أخرى
الانزيمات
Auxins
المؤلف:
J. Normanly, J. P. Slovin, and J. D. Cohen
المصدر:
Plant Physiol. 107, 323–329
الجزء والصفحة:
9-12-2015
4175
Auxins
1. History
Auxins were the first class of plant hormones to be discovered. Initial observations by Charles Darwin and his son Francis on the phenomenon of bending of canary grass coleoptiles toward a unilateral source of light (known as phototropism) led the foundation for a series of experiments that culminated in the discovery of the first phytohormone (1). The active substance was isolated from oat coleoptiles by Went in 1926 (2) and later identified and named auxin (from the Greek “to increase”). By analogy with the animal hormone concept from Bayliss and Starling (3), plant physiologists interpreted auxin as a phytohormone.
2. Biosynthesis and Metabolism
The most abundant natural auxin is indole-3-acetic acid (IAA). It is mainly synthesized in the shoot apex and in young leaves. Other substances with auxin activity that are found in plants are indole-3-butyric acid, 4-chloro-indole-acetic acid, and phenyl acetic acid (4, 5); these compounds are active only at higher concentrations, and their roles in development remain largely unknown. Multiple pathways exist for auxin biosynthesis; some of these are dependent on tryptophan, while others are independent (Fig. 1). In certain species, different routes are operational (6), whereas in others, essentially all of the IAA is derived from tryptophan (7). Three possible routes for tryptophan-dependent IAA synthesis have been proposed (4, 5), which include indole-3-acetonitrile, tryptamine, and indole-3-pyruvic acid as the respective intermediates. Genes encoding tryptophan decarboxylase) which catalyzes the conversion of tryptophan to tryptamine) and nitrilase (forming IAA from indole-3-acetonitrile) have been cloned (8-10). The route via indole-3-acetonitrile has been found primarily in Brassicaceae. Likewise, the tryptamine pathway is not supposed to be of general importance, because tryptamine is not universally present in plants. A unique tryptophan-dependent pathway via indole-3-acetamide is used by bacteria and by plant cells that are transformed with Agrobacterium tumefaciens. The corresponding genes have been used to alter the IAA levels in transgenic plants (11).
Figure 1. Auxin biosynthesis in higher plants. (Based on Ref. 4.)
Tryptophan-auxotroph mutants have revealed the existence of a tryptophan-independent pathway for IAA biosynthesis. Both maize (12) and Arabidopsis (13) tryptophan mutants overproduce IAA or IAA conjugates, respectively. On the other hand, mutants that block tryptophan biosynthesis before indole-3-glycerol phosphate have a phenotype suggestive of auxin deficiency (14). Based on these observations, IAA was proposed to be synthesized through a tryptophan precursor, either indole or indole-glycerol phosphate, thereby bypassing tryptophan (4). An auxin-overproducing mutant that accumulates both free and conjugated auxins has been isolated (15, 16). The wild-type gene product might inhibit auxin biosynthesis and shows similarity to aminotransferases (17).
Of all the auxin present in plant tissues, 95% resides in conjugated form, coupled to amino acids, peptides, or proteins via amide bonds, or to sugars via ester linkages (18). The role of these conjugates is diverse: the storage of free IAA (released by hydrolysis), its transport, and eventually its catabolism. A genetic approach was used to characterize a hydrolase that catalyzes the formation of free IAA from IAA-Leu (10). The ILR1 (IAA-leucine resistant 1) gene encodes a protein with similarity to a bacterial aminoacylase that catalyzes amide cleavage.
3. Transport
Another important aspect determining auxin levels is transport of the hormone. Auxin transport can be either (a) polar, basipetally directed, and active or (b) nonpolar and inactive (19). The latter occurs through phloem tissue. Polar transport is probably involved specifically in processes of apical dominance, vascular differentiation, and shoot–root communication. Evidence exists for the presence of influx and efflux carriers, although protonated auxin can enter cells by simple diffusion. Proton–auxin symport, energized by the plasma membrane proton pump, allows a more rapid uptake than by diffusion. The AUX1 protein has been suggested to represent an auxin uptake carrier (20), based on its similarity to amino acid uptake carriers, which also function as symporters. Although several mutants have been characterized that show growth defects similar to those upon treatment with polar transport inhibitors (21), only one of them appears to be a candidate for the auxin efflux carrier. The PIN1 (pin formed 1) gene product shows the characteristics of a transmembrane protein (22). The corresponding mutant displays abnormal cotyledon development, together with twisted, narrow leaves with branching midveins. Auxin transport is reduced at all stages of development (23).
Recently, factors that may modulate auxin transport have been characterized (24, 25). Both the loci RCN1 (ROOT CURLING on NPA1) (coding for a protein phosphatase A regulatory subunit) and MP1 (MONOPTEROS1) (coding for a homologue of the auxin response factor ARF1) (26) have been isolated based on their altered response to auxin transport inhibitors. Mutations in the MP1 gene interfere with vascular strand formation and with initiation of the body axis in the embryo (27).
4. Signal Perception and Transduction
The perception and transduction of the auxin signal remains largely enigmatic (Fig. 2). Two different approaches have been followed toward the identification of putative auxin receptors: (a) the characterization of auxin-resistant mutants and (b) the isolation of auxin-binding proteins (28, 29). Because auxin is present both intra- and extracellularly, a site of perception may be needed on both sides of the membrane. Although many auxin-binding proteins have been identified, none has been proven to function as an internal receptor at the molecular level. In contrast, an external receptor has been characterized. There is evidence that auxin causes changes in the plasma membrane potential via stimulation of the proton ATPase and modulation of ion channels. These rapid responses result from auxin binding to a protein named ABP1 (auxin-binding protein 1). Thus far, it is unknown whether ABP1 mediates cell growth. Although ABP1 possesses a C-terminal signal for retention in the endoplasmic reticulum (ER) and largely resides in the ER, it does not seem to bind auxin in this cellular compartment. Instead, ABP1 can reach the cell surface by a yet unknown mechanism, as demonstrated by immunolocalization experiments (30). It is hypothesized that ABP1 is retained at the membrane periphery by interaction with a docking protein, because ABP1 does not contain a potential membrane-spanning domain. The nature of the docking protein is undefined, but biochemical evidence supports the concept of a G-protein-coupled receptor (GCR) (31). These receptors contain seven transmembrane domains and are directly coupled to heterotrimeric G proteins. A possible effector could be phospholipase A2, which produces lysophospholipids and free fatty acids. The former have been proposed to act as second messengers that can activate the plasma membrane proton ATPase (32, 33). However, G-protein-coupled receptors may activate different G proteins in different cell types, as has been shown in animal cells (34), and therefore may control a downstream branched transduction pathway. At least one report suggests the involvement of a MAP kinase cascade in the initiation of cell division by auxin in cell cultures (35).
Figure 2. A model for auxin signal transduction. PL, phospholipids; PLA2, phospholipase A2; LPC, lysophospholipids; FA, fatty acids. (Modified from Refs. 29 and 39).
The second approach to molecular characterization of auxin signal transduction involves the isolation and characterization of auxin-resistant mutants. At least seven loci were identified by screening for resistance to auxin-induced root growth inhibition (21). AXR1 (auxin resistant 1(probably plays a role at an early stage in the signaling chain, because the recessive axr1 mutant cannot respond to auxin in all the assays tested. The AXR1 gene encodes a protein with homology to the amino-terminal half of the ubiquitin-activating enzyme E1 (36). Thus, a central part of the mechanism of auxin action appears to play a regulatory role in protein degradation. Recently, two genes of Saccharomyces cerevisiae were found to be similar to AXR1 (37). One of these regulates the stability of key components of cell cycle regulation, such as the cyclin-dependent kinase inhibitor Sic1p, through conjugation of the ubiquitin-like protein RUB1 to CDC53p, a component of a E3 ubiquitin—protein ligase complex (37). The speculation that AXR1 plays a role in cell cycle control is considerably strengthened by the characterization of the tir1 (transport inhibitor response 1(locus, a semidominant mutation that also confers auxin resistance (38). Sequence analysis revealed similarity between TIR1 and F-box proteins found in E3 ubiquitin-protein ligase complexes in yeast. The product of the SAR1 (suppressor of auxin resistance) gene has been proposed as a candidate target for AXR1-dependent ubiquitin degradation (39). The sar1 mutant was identified as a suppressor of the Axr1 phenotype (40). SAR1 may function as a repressor of AUX/IAA genes, or it may be involved in cell cycle regulation (40). The mostly root-specific axr4 mutation probably defines an AXR1-independent path. Double-mutant analysis indicates that at least two interacting pathways exist, in which AXR4 and AUX1 define a first route while AXR1 defines a second one (41) .
5. Downstream Targets
Ultimately, the auxin signal leads to induction or repression of a series of target genes, some of which are responsive in a few minutes (42, 43). The products of these primary response genes are likely candidates to play a role in auxin-stimulated growth. The induction of the early genes is independent of de novo protein biosynthesis, implying that post-translational processes control the activity of factors that affect their expression. The transcriptional activation of early genes is based on the presence of auxin-responsive, cis-acting, response elements (AuxREs). Two auxin response-element domains were defined in the Pisum sativum IAA4 and IAA5 genes, one of which acts as an auxin switch while the other acts as an enhancer element. The former AuxRE is present in the promoter of AUX/IAA, SAUR (small auxin-upregulated mRNAs, and auxin-inducible 1-aminocyclopropane-1-carboxylate synthase genes. An alternative AuxRE is found in the promoter of the GH2 and GH4 genes. Essentially, this element consists of a G-box motif, binds bZIP factors, and is generally responsive to stress signals. The biological role of most of the auxin early-response gene products remains to be elucidated. Nevertheless, based on similarities with proteins of known functions, it is proposed that GH2 and GH4 genes are glutathione-S-transferases. AUX/IAA polypeptide chains are short-lived nuclear proteins that probably function as an auxin switch in a secondary phase of signal transduction (42), implying that their target gene products probably mediate auxin responses. Thus, the AUX/IAA polypeptides might be involved in homo- and heterodimerization with auxin response factors, such as ARF1, that bind to AuxREs (26, 44). The IAA17 and AXR3 genes are identical (44). Axr3 is a dominant auxin-resistant mutant with extremely resistant roots, although the general phenotype of the mutant resembles auxin hypersensitivity (45).
6. Effects
Depending on temporal and spatial factors, a particular subset of downstream target genes is activated and results in one of many described effects. Auxin has been implicated in a variety of growth and developmental processes, such as cell division, stem and root elongation, leaf expansion, formation of adventitious and lateral roots, induction of vascular tissue, apical dominance, tropisms, flowering, and fertility (46).
References
1. C. Darwin and F. Darwin (1881) The Power of Movement in Plants, Appleton-Century-Crofts, New York.
2. F. W. Went (1927) Proc. Kon. Nederl. Akad. Wetensch. 30, 10–19.
3. W. M. Bayliss and E. H. Starling (1902) Proc. R. Soc. 69, 352–353.
4. J. Normanly, J. P. Slovin, and J. D. Cohen (1995) Plant Physiol. 107, 323–329.
5. B. Bartel (1997) Annu. Rev. Plant Physiol. Plant Mol. Biol. 48, 51–66.
6. L. Michalczuk, T. J. Cooke, and J. D. Cohen (1992) Phytochemistry 31, 1097–1103.
7. K. Bialek, L. Michalczuk, and J. D. Cohen (1992) Plant Physiol. 100, 509–517.
8. V. De Luca, C. Marineau, and N. Brisson (1989) Proc. Natl. Acad. Sci. USA 86, 2582–2586.
9. D. Bartling, M. Seedorf, A. Mithöferr, and E. W. Weiler (1992) Eur. J. Biochem. 205, 417–424.
10. B. Bartel and G. R. Fink (1995) Science 268, 1745–1748.
11. H. J. Klee and C. P. Romano (1994) Crit. Rev. Plant Sci. 13, 311–324.
12. A. D. Wright, M. B. Sampson, M. G. Neuffer, L. Michalczuk, J. P. Slovin, and J. D. Cohen (1991) Science 254, 998–1000.
13. R. L. Last, P. H. Bissinger, D. J. Mahoney, E. R. Radwanski, and G. R. Fink (1991) Plant Cell3 , 345-358 .
14. R. L. Last and G. R. Fink (1988) Science 240, 305–310.
15. W. Boerjan, M.-T. Cervera, M. Delarue, T. Beeckman, W. Dewitte, C. Bellini, M. Caboche, H. Van Onckelen, M. Van Montagu, and D. Inzé (1995) Plant Cell 7, 1405–1419.
16. J. J. King, D. P. Stimart, R. H. Fisher, and A. B. Bleecker (1995) Plant Cell 7, 2023–2037.
17. M. Gopalraj, T.-S. Tseng, and N. Olszewski (1996) Plant Physiol. 111 (Suppl.), 114 (469).
18. J. Normanly (1997) Physiol. Plant. 100, 431–442.
19. T. L. Lomax, G. K. Muday, and P. H. Rubery (1995) In Plant Hormones (P. J. Davies, ed.(, Kluwer Dordrecht, pp. 509–530.
20. M. J. Bennett, A. Marchant, S. T. May, H. G. Green, S. P. Ward, P. A. Millner, A. R. Walker, B. Schulz, and K. A. Feldmann (1996) Science 273, 948–950.
21. O. Leyser (1997) Physiol. Plant. 100, 407–414.
22. L. Galweiler, E. Wisman, A. Yephremov, and K. Palme (1996) P307
23. K. Okada, J. Ueda, M. K. Komaki, C. J. Bell, and Y. Shimura (1991) Plant Cell 3, 677–684.
24. C. Garbers, A. DeLong, J. Deruére, P. Bernasconi, and D. Söll (1996) EMBO J. 15, 2115–2124.
25. C. S. Hardtke and T. Berleth (1998) EMBO J. 17, 1405–1411.
26. T. Ulmasov, G. Hagen, and T. J. Guilfoyle (1997) Science 276, 1865–1868.
27. G. K. H. Przemeck, J. Mattsson, C. S. Hardtke, Z. R. Sung, and T. Berleth (1996) Planta 200, 229-237 .
28. H. Barbier-Brygoo (1995) Crit. Rev. Plant Sci. 14, 1–25.
29. H. Macdonald (1997) Physiol. Plant. 100, 423–430.
30. J. Deikman and M. Ulrich (1995) Planta 195, 440–449.
31. P. A. Millner, D. A. Groarke, and I. R. White (1996) Plant Growth Regul. 18, 143–147.
32. G. F. E. Scherer and B. André (1993) Planta 191, 515–523.
33. H. Yi, D. Park, and Y. Lee (1996) Physiol. Plant. 96, 359–368.
34. E. J. Neer (1995) Cell 80, 249–257.
35. T. Mizoguchi, Y. Gotoh, E. Nishida, K. Yamaguchi-Shinozaki, N. Hayashida, T. Iwasaki, H. Kamada, and K. Shinozaki (1994) Plant J. 5, 111–122.
36. H. M. O. Leyser, C. A. Lincoln, C. Timpte, D. Lammer, J. Turner, and M. Estelle (1993) Nature 364, 161-164 .
37. D. Lammer, N. Mathias, J. M. Laplaza, W. Jiang, Y. Liu, J. Callis, M. Goebl, and M. Estelle (1998) Genes Dev. 12, 914–926.
38. M. Ruegger, E. Dewey, W. M. Gray, L. Hobbie, J. Turner, and M. Estelle (1998) Genes Dev 12, 198-207 .
39. W. M. Gray and M. Estelle (1998) Curr. Opin. Biotechnol. 9, 196–201.
40. A. Cernac, C. Lincoln, D. Lammer, and M. Estelle (1997) Development 124, 1583–1591.
41. C. Timpte, C. Lincoln, F. B. Pickett, J. Turner, and M. Estelle (1995) Plant J. 8, 561–569.
42. S. Abel and A. Theologis (1996) Plant Physiol. 111, 9–17.
43. Y. Takahashi, S. Ishida, and T. Nagata (1995) Plant Cell Physiol. 36, 383–390.
44. D. Rouse, P. Mackay, P. Stirnberg, M. Estelle, and O. Leyser (1998) Science 279, 1371–1373.
45. H. M. O. Leyser, F. B. Pickett, S. Dharmasiri, and M. Estelle (1996) Plant J. 10, 403–413.
46. P. J. Davies (1995) Plant Hormones: Physiology, Biochemistry and Molecular Biology, Kluwer, Dordrecht, The Netherlands.
الاكثر قراءة في مواضيع عامة في الاحياء الجزيئي
اخر الاخبار
اخبار العتبة العباسية المقدسة
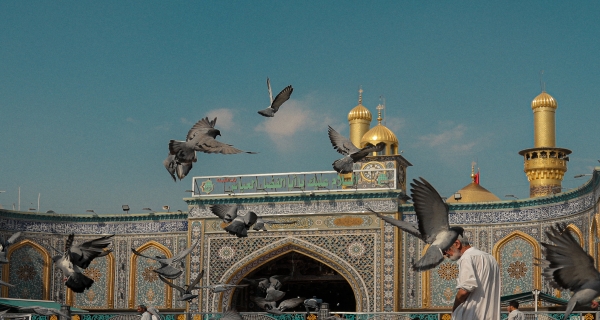
الآخبار الصحية
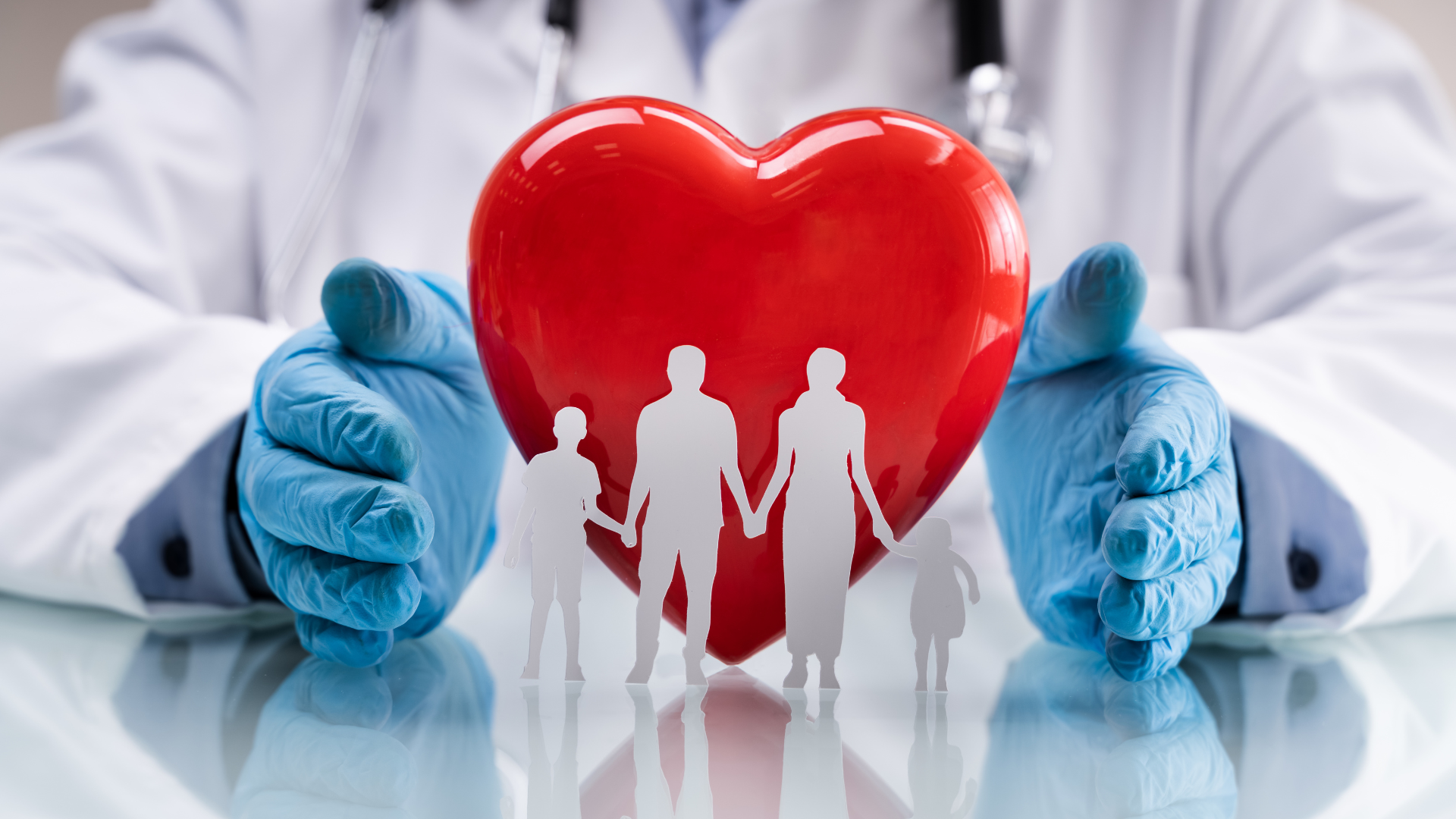