النبات
مواضيع عامة في علم النبات
الجذور - السيقان - الأوراق
النباتات الوعائية واللاوعائية
البذور (مغطاة البذور - عاريات البذور)
الطحالب
النباتات الطبية
الحيوان
مواضيع عامة في علم الحيوان
علم التشريح
التنوع الإحيائي
البايلوجيا الخلوية
الأحياء المجهرية
البكتيريا
الفطريات
الطفيليات
الفايروسات
علم الأمراض
الاورام
الامراض الوراثية
الامراض المناعية
الامراض المدارية
اضطرابات الدورة الدموية
مواضيع عامة في علم الامراض
الحشرات
التقانة الإحيائية
مواضيع عامة في التقانة الإحيائية
التقنية الحيوية المكروبية
التقنية الحيوية والميكروبات
الفعاليات الحيوية
وراثة الاحياء المجهرية
تصنيف الاحياء المجهرية
الاحياء المجهرية في الطبيعة
أيض الاجهاد
التقنية الحيوية والبيئة
التقنية الحيوية والطب
التقنية الحيوية والزراعة
التقنية الحيوية والصناعة
التقنية الحيوية والطاقة
البحار والطحالب الصغيرة
عزل البروتين
هندسة الجينات
التقنية الحياتية النانوية
مفاهيم التقنية الحيوية النانوية
التراكيب النانوية والمجاهر المستخدمة في رؤيتها
تصنيع وتخليق المواد النانوية
تطبيقات التقنية النانوية والحيوية النانوية
الرقائق والمتحسسات الحيوية
المصفوفات المجهرية وحاسوب الدنا
اللقاحات
البيئة والتلوث
علم الأجنة
اعضاء التكاثر وتشكل الاعراس
الاخصاب
التشطر
العصيبة وتشكل الجسيدات
تشكل اللواحق الجنينية
تكون المعيدة وظهور الطبقات الجنينية
مقدمة لعلم الاجنة
الأحياء الجزيئي
مواضيع عامة في الاحياء الجزيئي
علم وظائف الأعضاء
الغدد
مواضيع عامة في الغدد
الغدد الصم و هرموناتها
الجسم تحت السريري
الغدة النخامية
الغدة الكظرية
الغدة التناسلية
الغدة الدرقية والجار الدرقية
الغدة البنكرياسية
الغدة الصنوبرية
مواضيع عامة في علم وظائف الاعضاء
الخلية الحيوانية
الجهاز العصبي
أعضاء الحس
الجهاز العضلي
السوائل الجسمية
الجهاز الدوري والليمف
الجهاز التنفسي
الجهاز الهضمي
الجهاز البولي
المضادات الحيوية
مواضيع عامة في المضادات الحيوية
مضادات البكتيريا
مضادات الفطريات
مضادات الطفيليات
مضادات الفايروسات
علم الخلية
الوراثة
الأحياء العامة
المناعة
التحليلات المرضية
الكيمياء الحيوية
مواضيع متنوعة أخرى
الانزيمات
Group I Introns Undertake Self-Splicing by Transesterification
المؤلف:
JOCELYN E. KREBS, ELLIOTT S. GOLDSTEIN and STEPHEN T. KILPATRICK
المصدر:
LEWIN’S GENES XII
الجزء والصفحة:
20-5-2021
2824
Group I Introns Undertake Self-Splicing by Transesterification
KEY CONCEPTS
-The only factors required for autosplicing in vitro by group I introns are two metal ions and a guanosine nucleotide.
- Splicing occurs by two transesterification reactions, without requiring an input of energy.
- The 3′–OH end of the guanosine cofactor attacks the 5′ end of the intron in the first transesterification.
- The 3′–OH end generated at the end of the first exon attacks the junction between the intron and second exon in the second transesterification.
- The intron is released as a linear molecule that circularizes when its 3′–OH terminus attacks a bond at one of two internal positions.
- In Tetrahymena an internal bond of the excised intron can also be attacked by other nucleotides in a transsplicing reaction.
Group I introns are found in diverse species, and more than 2,000 of these introns have been identified to date. Unlike RNase P, group I introns are not essential for viability. Group I introns occur in the genes encoding rRNA in the nuclei of the unicellular/oligocellular eukaryotes T. thermophila (a ciliate) and Physarum polycephalum (a slime mold). They are common in the genes of fungi and protists, but are also found in prokaryotes, animals, bacteriophage, and viruses. Group I introns have an intrinsic ability to splice themselves. This is called autosplicing, or self-splicing. (This property also is found in the group II introns discussed in the section later in this chapter titled Group II Introns May Encode Multifunction Proteins.)
Self-splicing was discovered as a property of the transcripts of the rRNA genes in T. thermophila. The genes for the two major rRNAs follow the usual organization, in which both are expressed as part of a common transcription unit. The product is a 35S precursor RNA with the sequence of the small (17S) rRNA in the 5′ part and the sequence of the larger (26S) rRNA toward the 3′ end.
In some strains of T. thermophila, the sequence encoding the 26S rRNA is interrupted by a single, short intron. When the 35S precursor RNA is incubated in vitro, splicing occurs as an autonomous reaction. The intron is excised from the precursor and accumulates as a linear fragment of 400 bases, which is subsequently converted to a circular RNA. These events are summarized in FIGURE 1.
FIGURE .1 Splicing of the Tetrahymena 35S rRNA precursor can be followed by gel electrophoresis. The removal of the intron is revealed by the appearance of a rapidly moving small band. When the intron becomes circular, it electrophoreses more slowly, as seen by a higher band.
The reaction requires two metal ions and a guanosine nucleotide cofactor. No other base can be substituted for G, but a triphosphate is not needed: GTP, GDP, GMP, and guanosine itself all can be used, indicating that there is no net energy requirement. The guanosine nucleotide must have a 3′–OH group.
The fate of the guanosine nucleotide can be followed by using a radioactive label. The radioactivity initially enters the excised linear intron fragment. The G residue becomes linked to the 5′ end of the linear intron by a normal phosphodiester bond. FIGURE .2 shows that three transfer reactions occur. In the first transfer, the guanosine nucleotide behaves as a cofactor providing a free 3′–OH group that attacks the 5′ end of the intron. This reaction creates the G–intron link and generates a 3′–OH group at the end of the 5′ exon (labeled Exon A). The second transfer involves a similar chemical reaction, in which the newly formed 3′–OH at the end of Exon A attacks Exon B. The two transfers are connected; no free exons have been observed, so their ligation may occur as part of the same reaction that releases the intron. The intron is released as a linear molecule, but the third transfer reaction converts it to a circle.
FIGURE .2 Self-splicing occurs by transesterification reactions in which bonds are exchanged directly. The bonds that have been generated at each stage are indicated by the blue circles.
Each stage of the self-splicing reaction occurs by a transesterification, in which one phosphate ester is converted directly into another without any intermediary hydrolysis. Bonds are exchanged directly and energy is conserved, so the reaction does not require input of energy from hydrolysis of ATP or GTP. Each consecutive transesterification reaction involves no net change of energy. In the cell, the concentration of GTP is high relative to that of RNA, and therefore drives the reaction forward. Under physiological conditions, this reaction is essentially irreversible, allowing the reaction to proceed to completion.
The ability to splice is intrinsic to the RNA, and the system is able to proceed in vitro without addition of any protein components. The RNA forms a specific secondary/tertiary structure in which the relevant groups are brought into juxtaposition so that a guanosine nucleotide can be bound to a specific site and then the bond breakage and reunion reactions shown in Figure .2 can occur.
Although a property of the RNA itself, the reaction is very slow in vitro. This is because group I intron splicing is assisted in vivo by proteins that serve to stabilize the RNA structure in a favorable conformation for splicing.
The ability to engage in these transfer reactions resides with the sequence of the intron, which continues to be reactive after its excision as a linear molecule. FIGURE .3 summarizes catalytic activities of the excised intron from Tetrahymena, with residue numbers corresponding to that organism.
FIGURE .3 The excised intron can form circles by using either of two internal sites for reaction with the 5′ end and can reopen the circles by reaction with water or oligonucleotides.
The intron can circularize when the 3′ terminal G (ΩG) attacks an internal position near the 5′ end. The internal bond is broken and the new 5′ end is transferred to the 3′–OH end of the intron, circularizing the intron. The previous 5′ end with the original exogenous guanosine nucleotide (exoG) is released as a linear fragment (not shown). The circularized intron can be linearized by specifically hydrolyzing the bond between ΩG and the internal residue that had closed the circle. This is called a reverse cyclization. Depending on the position of the primary cyclization, the linear molecule generated by hydrolysis remains reactive and can perform a secondary cyclization.
The final product of the spontaneous reactions following release of the Tetrahymena group I intron is the L-19 RNA, a linear molecule generated by reversing the shorter circular form. This molecule has an enzymatic activity that allows it to catalyze the extension of short oligonucleotides. The reactivity of the released intron extends beyond merely reversing the cyclization reaction. Addition of the oligonucleotide UUU reopens the primary circle by reacting with the ΩG–internal nucleotide bond. The UUU (which resembles the 3′ end of the 15-mer released by the primary cyclization) becomes the 5′ end of the linear molecule that is formed. This is an intermolecular reaction, and thus demonstrates the ability to connect two different RNA molecules.
This series of reactions demonstrates vividly that the autocatalytic activity reflects a generalized ability of the RNA molecule to form an active center that can bind guanosine cofactors, recognize oligonucleotides, and bring together the reacting groups in a conformation that allows bonds to be broken and rejoined. Other group I introns have not been investigated in as much detail as the Tetrahymena intron, but their properties are generally similar.
The autosplicing reaction is an intrinsic property of RNA in vitro, but many appear to require proteins in vivo. Some indications for the involvement of proteins are provided by mitochondrial systems, where splicing of group I introns requires the trans-acting products of other genes. One striking case is presented by the cyt18 mutant of Neurospora crassa, which is defective in splicing several mitochondrial group I introns. The product of this gene turns out to be the mitochondrial tyrosyl-tRNA synthetase. This is explained by the fact that the intron can take up a tRNA-like tertiary structure that is stabilized by the synthetase, thereby promoting the catalytic reaction. This relationship between the synthetase and splicing is consistent with the idea that splicing originated as an RNAmediated reaction, subsequently assisted by RNA-binding proteins that originally had other functions. The in vitro self-splicing ability may represent the basic biochemical interaction. The RNA structure creates the active site, but is able to function efficiently in vivo only when assisted by a protein complex.
الاكثر قراءة في مواضيع عامة في الاحياء الجزيئي
اخر الاخبار
اخبار العتبة العباسية المقدسة
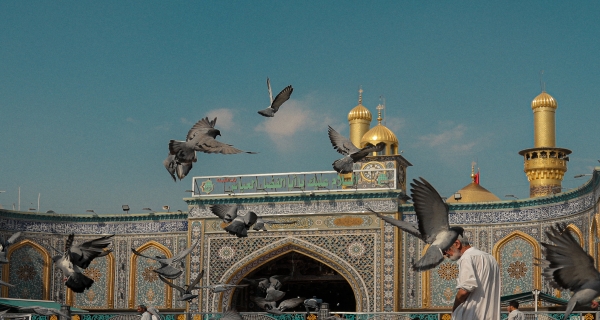
الآخبار الصحية
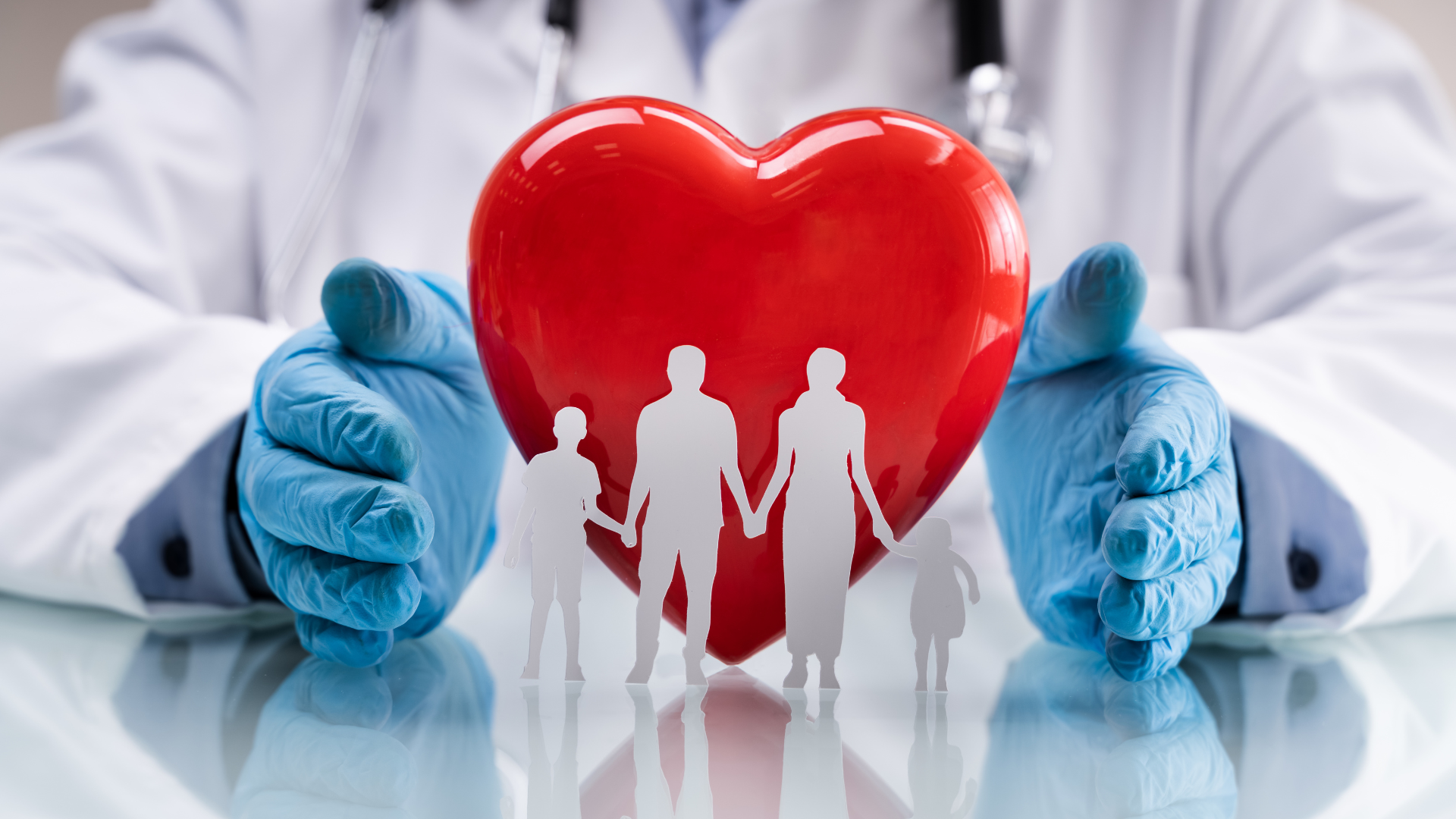